Ⅰ. Introduction
Global seafood consumption has risen exponentially over the past years, with consumption levels estimated to reach approximately 130 million tonnes in the year 2010 (1). Seafood is mainly classified into fish, mollusca, crustacean and aquatic animals and plants. Even though the consumption of seafood has been associated with various health and nutritional benefits, it also comes with considerable amount of risk. One of the significant risks is the presence of microbial pathogens which is responsible for the occurrence of a large percentage of foodborne outbreaks. According to data collated by Iwamoto et al. (2) for the period 1973~2006, there was a general increase in the number of foodborne outbreaks related to seafood. Furthermore, it was also shown that bacterial pathogens were responsible for most of these foodborne outbreaks.
The associated risk contamination is dependent on the type of seafood. Accumulation of bacterial pathogen may occur to a greater extent in certain mollusca species which are filter-feeders. Consumption risk is also elevated as these foods are usually consumed raw or cooked under mild conditions. This risk was highlighted by Iwamoto et al. (2) who showed that there was an increasing trend in the percentage of foodborne outbreaks related to mollusca compared to other seafood types in a 4 multi-year period.
In this review, the main routes of contamination and pathogens associated with seafood products are first highlighted. Biological and chemical intervention technologies employed to reduce these pathogens in seafood products are then discussed.
Ⅱ. Sources of Contamination
Contamination of seafood with bacterial pathogens could mainly originate from the source and cross-contamination along the post-harvest processing line. Foodborne illnesses can occur when seafood products are consumed in large quantities such that the effective infectious dose of the respective pathogens has been exceeded. At the source, bacterial pathogens could exist naturally within the water source or introduced via human means such as discharge from sewage treatment plants or marine vessels.
Gram-positive and −negative bacteria such as Vibrio spp., Aeromonas spp, Shigella spp. and spores of type F Clostridium botulinum are common pathogens that are found within water sources and are responsible for causing diseases such as gastroenteritis as well as severe diarrheal, urinary tract and fever symptoms. It was observed that cases of foodborne illness attributing to Vibrio spp. were commonly associated with consumption of seafood (2). Fecal material are common reservoirs for pathogens belonging to Salmonella, Vibrio, Shigella and Campylobacter genus as well as enterotoxin-forming bacteria such as C. perfringens and Bacillus cereus and they can also be introduced into water sources when sewage discharge are not properly regulated.
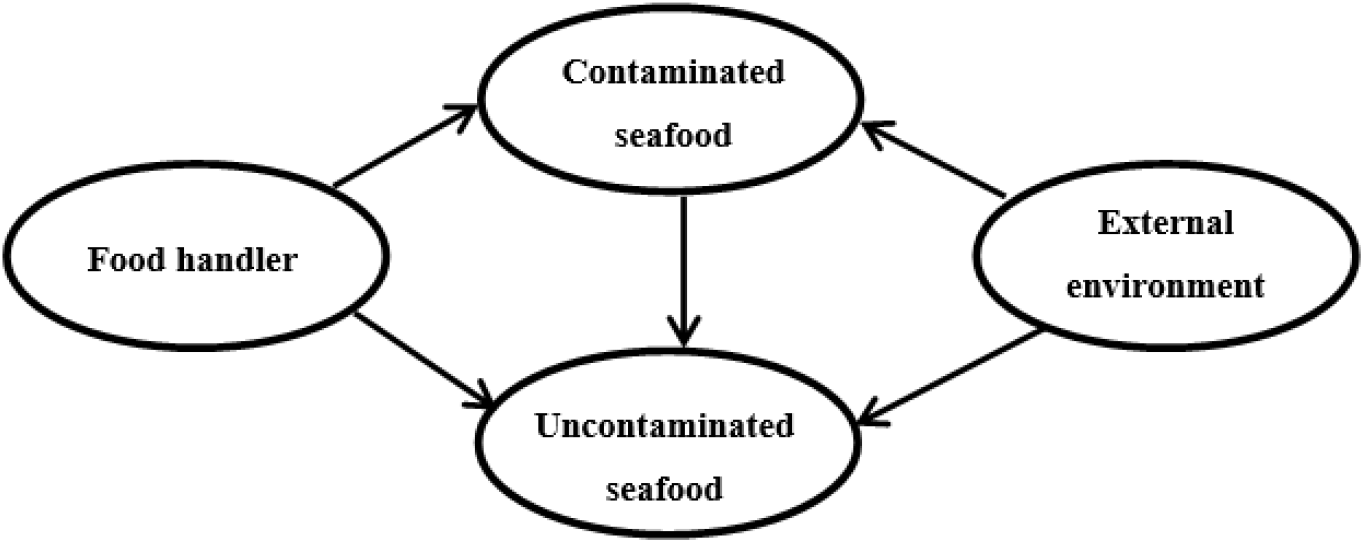
Following harvesting, cross-contamination is another source contributing to pathogens present in seafood. Figure 1 shows the interactions between the food-handler, contaminated seafood, environment and uncontaminated seafood that affect safety of seafood consumption. Cross-contamination can occur from the food handler or the processing equipment to contaminated or uncontaminated seafood. Contaminated seafood can also contaminate subsequent batches of seafood which may not have been previously contaminated. Furthermore, the risk from such initial contamination could substantially be increased when seafood products are not stored or processed under appropriate conditions. As a result, this may cause bacterial pathogens to accumulate to infectious levels and in turn, result in food-borne outbreak upon consumption. Therefore, regulatory agencies in different countries have laid down regulations in order to increase safety in seafood consumption.
Ⅲ. Biological Intervention Technologies
Bacteriocins are proteins or protein complexes that exert antimicrobial activity by adversely affecting membranes, DNA synthesis and protein synthesis (3). They are produced by lactic acid bacteria (LAB), and the relevant bacteriocinogenic LAB in seafood are Carnobacterium and Enterococcus (3). Bacteriocins can be applied to food directly as isolated bacteriocins or indirectly as bacteriocinogenic starter culture. Nisin is the best-studied bacteriocin and is permitted in more than 50 countries, including USA and some European countries (3), while licensed commercial bacteriocinogenic starter cultures, such as Alt 2341 (Quest BioTechnology Inc., Sarasota, FL, USA), for use in food industry are also available.
When applied in seafood products, bacteriocins have been shown to be effective against L. monocytogenes (4–8) and Vibrio spp. (6, 9). Work has also been done on isolating bacteriocins produced by bacteria found naturally in seafood or water samples (6, 9, 10). This offers the advantage of ensuring that the bacteriocinogenic microorganism can survive in seafood matrix. Additionally, it was observed that simultaneous application of different bacteriocins resulted in greater antimicrobial effectiveness, and thus it was suggested that LAB which produced multiple bacteriocins have greater potential as biopreservatives (5, 6).
However, when bacteriocins are applied directly to food, their effectiveness may be affected by their stability, solubility, diffusivity, distribution and release from food matrix. Being protein in nature, bacteriocins can be degraded by enzymes or interact with food or packaging molecules (11). Strong adsorption of the bacteriocins to the food matrix also reduces their antimicrobial efficacy (7). To overcome these problems, bacteriocins can be incorporated into packaging materials instead to allow its sustained release throughout storage period, and its potential has been demonstrated in the study by Neetoo et al. (12), which observed a reduction of 3.9 log CFU/cm2 of L. monocytogenes in vacuum-packed cold-smoked salmon, although the migration of nisin is temperature- and pH-dependent.
Using only isolated bacteriocins on foods increases the likelihood of target bacteria developing resistance (7), and hence the use of bacteriogenic LAB as starter culture was proposed as a more effective means due to additional antimicrobial mechanisms offered by LAB (11). Still, this may be problematic, as the amount of bacteriocin produced by LAB could be low, possibly due to repression of bacteriocin synthesis, lack of induction factors and influence of environmental factors, such as the environmental pH and the presence of additives (7). Such implications should be considered when using bacteriocins as biopreservatives.
Generally, there is minimal deterioration in organoleptic qualities when bacteriocins are applied in seafood products, although careful selection of LAB strains used is needed to ensure that they do not produce high levels of biogenic amines, which could give off-odors. Nykänen et al. (8) observed that use of nisin with sodium lactate on cold-smoked rainbow trout did not cause significant changes in the odor, flavor and texture, while Weiss and Hammes (2006) noted that the pH and sensorial properties of cold-smoked salmon were unaffected by inoculation of bacteriocinogenic L. sakei TH4122 and 5754. Additionally, the possible differences in the use of divergicin M35 and C. divergens strain (M35 bacteriocin and its starter culture, respectively) on smoked salmon were investigated by Tahiri et al. (13), and they showed that although there were differences between the two treatments in terms of texture, color and total volatile base nitrogen production, these differences were not perceivable in sensory evaluation of the treated samples. The salmon slices from both treatments also showed better color, odor characteristics and firmer texture relative to the control after 21 storage days, underlining the potential of these bacteriocin and LAB culture for practical use.
LAB are common bacteria used to suppress the growth of spoilage or pathogenic microorganisms through their antagonistic effects. In fresh and sea water fresh fish, the associated LAB genera are Carnobacterium, Enterococcus, Lactobacillus and Lactococcus. In lightly preserved fish products which are packed in vacuum or modified atmosphere, the dominant LAB are Carnobacterium, Lactobacillus, Lactococcus and Leuconostoc (14, 15). The principal LAB species isolated from lightly preserved seafood products has been summarized by Françoise (14). Currently, the use of selected generic LAB as biopreservatives is allowed and regulated by the FDA under GRN No.000171 (16), although their use is not typically applied in seafood products. Most of the LAB protective cultures isolated from seafood have not been approved for use in the EU (3).
LAB is antagonistic to spoilage and pathogenic bacteria such as Listeria, Clostridium, Staphylococcus, and Bacillus spp. through competition for nutrients and/or action of its antimicrobial metabolites, such as organic acids, hydrogen peroxide, fatty acids, carbon dioxide and bacteriocins (15). Lactic and acetic acids are the common organic acids produced by LAB. Other than reducing environmental pH, undissociated organic acids can penetrate into the bacterial cell, reducing intracellular pH, denaturing proteins and disrupting cell metabolism (17). Hydrogen peroxide exerts antimicrobial effect by lipid oxidation of cell membrane components, while other products such as fatty acids and carbon dioxide generally lead to unfavorable environmental conditions for growth of spoilage and pathogenic bacteria (17). Some LAB also produce bacteriocins, which are proteins or protein complexes with selective bactericidal activity as described above. These metabolic compounds can act synergistically to result in an enhanced antimicrobial effect.
The main advantage of using seafood-borne LAB is that they are compatible with the storage environment of seafood products: they can grow at refrigerated temperatures, under modified atmosphere package (MAP), low pH, high salt concentration conditions, and in presence of additives like lactic or acetic acid (18, 20). The anti-bacterial effectiveness and inhibition spectrum of some seafood-borne LAB isolates have studied, and these LAB have been shown to be effective in inhibiting growth of typical seafood spoilage and pathogenic bacteria, including L. monocytogenes and E. coli (19, 21). Other than introducing LAB in seafood products, LAB could also be used for depuration purposes. Xi et al. (22) demonstrated that the addition of L. plantarum in depuration of pacific oyster samples improved microbial load reduction of V. parahaemolyticus. It was proposed that lactic acid produced by LAB had altered oyster membrane properties and increased uptake of antibacterial compounds, leading to greater microbial inhibition. Alternatively, competitive exclusion due to LAB growth could have also caused reduction in the population of V. parahaemolyticus.
However, certain LAB could also potentially generate off-flavor, odors and bitterness due to organic acid production, protein degradation and amino acid degradation respectively (23). The organoleptic impact of LAB on seafood products such as cold-smoked salmon has been studied, and it was generally concluded that whether or not LAB act as spoilage organisms depends on the species and cell load (21, 25, 26). Nutritional quality could also possibly be adversely affected. Furthermore, while LAB have been shown to be effective in liquid media (19, 24), this could vary in seafood products depending on the LAB species used. The low-carbohydrate and slightly acidic environment common in most seafood products is unfavorable for establishment of certain LAB, and can lead to reduced effectiveness (27, 28).
Given the relatively narrow range of food environment which LAB function in, their application becomes limited and case-by-case consideration is required. Hence, despite promising results of LAB in lab studies, their use is less common in seafood products compared to dairy and meat products. Further investigation is needed on the use of seafood-isolated LAB in food matrices, the potential undesirable effects on organoleptic and nutritional quality, and their compliancy with the regulatory requirements.
Other than using LAB on harvested seafood products, LAB can be used as a pre-harvest microbiological control to improve water quality and reduce growth of commensal pathogens and spoilage microorganisms in the guts of fishes and shellfish. The antimicrobial mechanism of LAB as probiotics (used in live seafood) thus differs slightly from that as biopreservatives (used in dead seafood). Compared to conventional methods, the use of probiotics does not lead to the development of antibiotic-resistance in animals nor significantly affects the organoleptic quality of food (29), therefore allowing the production of quality raw seafood safe for consumption.
Inhibition of bacteria by probiotics in animals have been suggested to be due to several mechanisms: competitive exclusion of pathogens, enhanced nutrient absorption, direct uptake of dissolved organic matter, improved immunity against pathogens, and production of antibacterial molecules like bacteriocins to exert a direct inhibition effect (29, 30). Probiotic strains have been isolated from the intrinsic microbiota of aquatic animals, and most of the probiotics used in studies are from the genus Lactobacillus, Carnobacterium, Vibrio, Bacillus and Pseudomonas, as previously reviewed by Balcázar et al. (29).
In general, aquatic animals treated with probiotics showed increased resistance to Streptococcosis, Lactococcosis, Furunculosis, Vibriosis, fin rot and other diseases. Most of the probiotics which were shown to be effective were isolated from aquatic animals and water samples. Although the use of probiotic strains which are already approved for use in human seems more likely to garner greater consumer acceptance, a study by Nikoskelaninen (31) showed that the selected six probiotic strains safe for human use had limited effectiveness in inhibiting A. salmonicida, a disease-causing agent, in the gut of rainbow trout (31). Presently, microorganisms approved for use in animal feed are chiefly from the genera Bacillus, Enterococcus, Lactobacillus, Pediococcus, and Streptococcus in the EU, while none has been approved yet in the US and Japan. The main methods of probiotics introduction to aquatic farms are through direct addition to feed, enrichment of live food and addition to water, and it was suggested that the supply of probiotics should be sustained as any beneficial effect are easily reverted on stopping (30). Despite promising studies, probiotics are still not used in aquatic farming commercially. The lack of adequate knowledge on the mechanisms of probiotics in seafood complicates the selection process for potential probiotics. More understanding is also required to allow better consideration of the impact of LAB on ecosystems.
The potential of using bacteriophages in the washing of raw seafood or direct application in ready-to-eat seafood products have been demonstrated, as summarized in Table 1. A few phages, such as ListexTMP1000 and ListShieldTM, have also been legalized for use in various countries and they have been shown to be effective with minimal impact on organoleptic qualities.
The effectiveness of phages depends on several factors, including the phage concentration, food matrix and storage conditions. Phages immobilized by the food matrix cannot diffuse and infect bacteria cells, and this could result in the reduced efficacy of listeriophages applied to smoked salmon and ready-to-eat seafood (32). The high specificity of phages also limits its use, and mixtures of different phages or broad-host-range phages are more commonly used (32). Additionally, Vibrio spp. has been shown to escape phage recognition and infection by changing its surface structures (33). These drawbacks limit the use of phages in seafood products.
Ⅳ. Conclusion
The rising demand for high-quality seafood products and the popularity of consuming raw seafood has driven the development of various emerging intervention technologies in seafood processing. Current technologies which have been approved for industrial use include bacteriocins and bacteriophages, and they have been shown to effectively reduce microbial load in seafood products without adversely affecting their quality. On the other hand, research has been ongoing on several other novel preservation techniques, which include use of LAB and probiotics. Despite promising results demonstrated in scientific studies, they are yet to be approved for industrial use due to various reasons as elaborated previously. Further work can be done on investigation of scale-up issues, optimization of parameters, and understanding of the possible safety and environmental concerns related to these technologies. This allows more insightful and complete evaluation of these technologies such that their use can be approved and regulated in the industry. Lastly, as the combined use of technologies has been observed to exert synergistic effects, more effort can be focused on this area such that the freshness and quality of seafood can be maintained without applying excessively harsh treatments.